17 May 2024
PLEASE NOTE: The Viewpoints on our website are to be read and freely shared by all. If they are republished, the following text should be used: “This Viewpoint was originally published on the REVIVE website revive.gardp.org, an activity of the Global Antibiotic Research & Development Partnership (GARDP).”
The views and opinions expressed in this article are solely those of the original author(s) and do not necessarily represent those of GARDP, their donors and partners, or other collaborators and contributors.
The current antibiotic development pipeline is broken, with a lack of pull incentives for pharmaceutical companies to continue to bring new antibiotics from the bench to the bedside. This has helped fuel the antibiotic resistance crisis. This crisis is often referred to as a slow-moving pandemic, however, this is a misnomer, as the impacts are already being felt in almost every hospital around the world, as patients succumb to infections that frontline antimicrobial therapies fail to clear. Indeed, mortality levels associated with antimicrobial resistance are as high as those attributed to climate change,1,2 yet the national and international political and financial agendas fail to reflect this parity. The failure of antibiotic development pipelines to date is driving a renewed interest in exploring alternatives to traditional antibiotic modalities. For example, phage therapy is now being actively explored at governmental level in the UK and in many other countries.3 Another alternative strategy to tackle multidrug resistance (MDR) is the targeted disruption of virulence factors essential for infection using next-generation antimicrobials (NGAs).
The repeated failures of the traditional antibiotic development pipelines are corroding more than a century of medical progress with almost all aspects of medicine under threat.
Targeting the virulence of bacteria
Traditional antibiotic discovery aims to identify bactericidal or bacteriostatic compounds that impact growth. However, as evidenced time and time again in the clinic, this imposes a strong selective pressure for the evolution of resistant strains. Anti-virulence compound discovery aims to identify disruptors of the key mechanisms that facilitate host colonisation without impacting bacterial growth, for example, if a pathogen can no longer produce tissue-damaging toxins then it becomes a much less efficient pathogen. This strategy can give the immune system time to mount a robust immune response and also has the capacity to increase pathogen susceptibility to frontline antibiotics.4 The concept of targeting bacterial virulence and in particular bacterial toxins as a means to limit infection progression is not new. In 1901, von Behring and Kitasato earned a Nobel prize for their work successfully demonstrating the effectiveness of antibody-based anti-toxins against Corynebacterium diphtheriae toxin and Clostridium tetani toxin.5 However, with the subsequent advent of antibiotics, the interest and perceived need for such approaches waned, with only one other antibody-based anti-virulence treatment being approved for clinical use.6 The impacts of the antibiotic resistance crisis are now forcing a deeper exploration of the clinical potential of anti-virulence approaches.
Disrupting biofilm formation
One particular virulence phenotype that has emerged as a potent target for NGA development is biofilm formation. Bacteria can attach to each other or a surface and encase themselves in a matrix composed of polysaccharides, extracellular DNA (eDNA) and proteins, forming a biofilm.7 In doing so, bacteria are more tolerant to chemical disinfectants, the host immune system and are estimated to be 10–1,000 times more tolerant to antibiotics than their planktonic counterparts. Indeed, upwards of 80% of chronic infections are associated with bacterial biofilms.8
Targeting the different components of a biofilm has been repeatedly shown to be an effective means to prevent biofilm formation or to disrupt an established biofilm. eDNA is the rebar scaffold that maintains biofilm structural integrity.9 Disrupting this scaffold using DNases effectively reduces bacterial biofilms and improves infection outcomes in a range of in vivo models.10 Clinically, DNases are used to loosen the mucus in the lungs of cystic fibrosis (CF) patients. However, a common co-morbidity of CF patients is chronic biofilm-associated bacterial infections. In vitro assays have demonstrated that DNases can disrupt biofilms formed by CF-associated pathogens indicating that the use of DNases is likely having a dual effect, loosening mucus and disrupting biofilms.11
The widely recognised urgent need to develop novel strategies to tackle MDR pathogens has meant there is now a global onus to accelerate the clinical development of non-traditional approaches such as targeting virulence.
Equally targeting exopolysaccharides using hydrolases and extracellular proteins using proteases have both been shown to improve infection outcomes in in vivo models and, in some instances, improve the efficacy of antibiotics.11 Targeting a pathogen’s capacity to adhere to the host is also another promising strategy to limit or prevent colonisation. The cell surface of bacterial pathogens is decorated with a range of receptors that can facilitate attachment to the host. Preventing the assembly of these receptors or blocking them using molecular mimicry has shown significant potential in a range of animal models.12 Antibiofilm peptides have also emerged as a particularly promising means to target biofilms with examples of synthetic and natural peptides having been identified that can target eDNA, adhesion, exopolysaccharide production, the stringent response and motility.13 Many of these peptides exhibit these activities without impacting bacterial growth or at concentrations that do not impact growth, lessening the probability of resistance and classifying them as NGAs.
Disrupting quorum sensing
Bacteria coordinate their behaviours using a suite of global virulence-associated regulatory pathways. Genetic studies have shown that disrupting these pathways can render a pathogen avirulent.14 One of the most well-targeted virulence-associated regulatory pathways is quorum sensing (QS).15 This system allows bacteria to determine the local population through the production of extracellular signalling molecules termed autoinducers. When this autoinducer reaches a threshold density, it binds to its cognate response regulator triggering a range of virulence-associated phenotypes, such as toxin production, motility and biofilm formation. Ecologically, disrupting QS is an effective bacterial mechanism to outcompete other species in a given ecosystem, underlying the clinical potential of such a strategy.16 Indeed, many of the compounds and enzymes that have been identified to disrupt QS are derived from other bacteria or plants, which have over millennia evolved effective mechanisms to secure a favourable ecological niche or prevent pathogen colonisation.14 Despite a wealth of compelling in vivo studies and evidence from clinical trials supporting the therapeutic potential of QS-disrupting compounds,17,18 the clinical potential of this approach has yet to be realised. In light of the ever-increasing pressures being applied by MDR infections, this is perhaps an area that needs to be supported with greater translational vigour.
Mortality levels associated with antimicrobial resistance are as high as those attributed to climate change, yet the national and international political and financial agendas fail to reflect this parity.
The widely recognised urgent need to develop novel strategies to tackle MDR pathogens has meant there is now a global onus to accelerate the clinical development of non-traditional approaches such as targeting virulence (Figure 1), particularly as a precedent for the potential of this approach has already been set with the successful clinical use of antibody-based anti-toxins.5,6 To further facilitate the clinical development of anti-virulence compounds, we need to know more about the capacity for pathogens to evolve resistance to their activity in vitro and in vivo, as although there is less acute selection for resistance, it can still occur. It will also be important to explore how these anti-virulence compounds, if given in combination with traditional antibiotics, impact the evolution of antibiotic resistance.
Figure 1: A summary of the most studied targets of anti-virulence drug discovery
The impact that anti-virulence compounds can have on the host and the host commensal microbiome is another key consideration. Interestingly however, many compounds that display potent anti-virulence potential can be found in the human diet, and as a result much is known about their impact on the host, the microbiome and about their pharmacokinetics and pharmacodynamics within the body, which will undoubtedly help accelerate the clinical development of such compounds either as standalone therapies or as adjuvants for traditional antibiotics.17,19,20 Strikingly, despite the clinical potential of compounds targeting virulence, there are only 24 antimicrobials (11%) in the preclinical pipeline that have anti-virulence properties,21 which suggests greater support is needed to bridge the gap between compelling in vivo lab data and clinical use. There also needs to be a clearer regulatory framework for the licencing specifically of anti-virulence-based therapies developed to support this transition.
The repeated failures of the traditional antibiotic development pipelines are corroding more than a century of medical progress with almost all aspects of medicine under threat. Targeting virulence rather than viability represents an alternative strategy which will have an increased clinical importance in the coming years if we are to prevent the devastating consequences of antibiotic resistance.
References
- Murray CJ, Ikuta KS, Sharara F, Swetschinski L, Aguilar GR, Gray A, Han C, Bisignano C, Rao P, Wool E, Johnson SC. (2022) Global burden of bacterial antimicrobial resistance in 2019: a systematic analysis. The Lancet. 399(10325):629-55.
- Zhao Q, Guo Y, Ye T, Gasparrini A, Tong S, Overcenco A, Urban A, Schneider A, Entezari A, Vicedo-Cabrera AM, Zanobetti A. (2021) Global, regional, and national burden of mortality associated with non-optimal ambient temperatures from 2000 to 2019: a three-stage modelling study. The Lancet Planetary Health. 5(7):e415-25.
- UK House of Commons (2024) The antimicrobial potential of bacteriophages [Accessed 16/05/24]
- Gadar K, McCarthy RR. (2023) Using next generation antimicrobials to target the mechanisms of infection. npj Antimicrobials and Resistance. 1(1):11.
- Kantha SS. (1991) A centennial review; the 1890 tetanus antitoxin paper of von Behring and Kitasato and the related developments. The Keio journal of medicine. 40(1):35-9.
- Alonso CD, Mahoney MV. (2018) Bezlotoxumab for the prevention of Clostridium difficile infection: a review of current evidence and safety profile. Infection and drug resistance. 17:1-9.
- Sauer K, Stoodley P, Goeres DM, Hall-Stoodley L, Burmølle M, Stewart PS, Bjarnsholt T. (2022) The biofilm life cycle: Expanding the conceptual model of biofilm formation. Nat Rev Microbiol. 20(10):608-620.
- Sharma D, Misba L, Khan AU. (2019) Antibiotics versus biofilm: an emerging battleground in microbial communities. Antimicrobial Resistance & Infection Control. 8(1):1-0.
- Whitchurch CB, Tolker-Nielsen T, Ragas PC, Mattick JS. (2002) Extracellular DNA required for bacterial biofilm formation. Science. 295(5559):1487-.
- Fleming D, Rumbaugh KP. (2017) Approaches to dispersing medical biofilms. Microorganisms. 5(2):15.
- Kaplan JB, LoVetri K, Cardona ST, Madhyastha S, Sadovskaya I, Jabbouri S, Izano EA. (2012) Recombinant human DNase I decreases biofilm and increases antimicrobial susceptibility in staphylococci. The Journal of antibiotics. 65(2):73-7.
- Krachler AM, Orth K. (2013) Targeting the bacteria–host interface: strategies in anti-adhesion therapy. Virulence. 15;4(4):284-94.
- Hancock REW, Alford MA, Haney EF. (2021) Antibiofilm activity of host defence peptides: complexity provides opportunities. Nat Rev Microbiol. 19(12):786-797.
- Pearson JP, Feldman M, Iglewski BH, Prince A. (2000) Pseudomonas aeruginosa cell-to-cell signaling is required for virulence in a model of acute pulmonary infection. Infection and immunity. 68(7):4331-4.
- Soukarieh F, Williams P, Stocks MJ, Camara M. (2018) Pseudomonas aeruginosa quorum sensing systems as drug discovery targets: current position and future perspectives. Journal of medicinal chemistry. 61(23):10385-402.
- Rémy B, Mion S, Plener L, Elias M, Chabrière E, Daudé D. (2018) Interference in bacterial quorum sensing: a biopharmaceutical perspective. Frontiers in pharmacology. 9:203.
- Smyth AR, Cifelli PM, Ortori CA, Righetti K, Lewis S, Erskine P, Holland ED, Givskov M, Williams P, Cámara M, Barrett DA. (2010) Garlic as an inhibitor of Pseudomonas aeruginosa quorum sensing in cystic fibrosis—a pilot randomized controlled trial. Pediatric pulmonology. 45(4):356-62.
- Jonkergouw, C., Beyeh, N.K., Osmekhina, E., Leskinen, K., Taimoory, S.M., Fedorov, D., Anaya-Plaza, E., Kostiainen, M.A., Trant, J.F., Ras, R.H. and Saavalainen, P. (2023) Repurposing host-guest chemistry to sequester virulence and eradicate biofilms in multidrug resistant Pseudomonas aeruginosa and Acinetobacter baumannii. Nature Communications, 14(1), p.2141.
- Gadar K, de Dios R, Kadeřábková N, Prescott TA, Mavridou DA, McCarthy RR. (2023) Disrupting iron homeostasis can potentiate colistin activity and overcome colistin resistance mechanisms in Gram-Negative Bacteria. Communications Biology. 6(1):937.
- de Dios R, Proctor CR, Maslova E, Dzalbe S, Rudolph CJ, McCarthy RR. (2023) Artificial sweeteners inhibit multidrug‐resistant pathogen growth and potentiate antibiotic activity. EMBO Molecular Medicine. 15(1):e16397.
- World Health Organization (2024) WHO antibacterial preclinical pipeline review [Accessed 16/05/24]
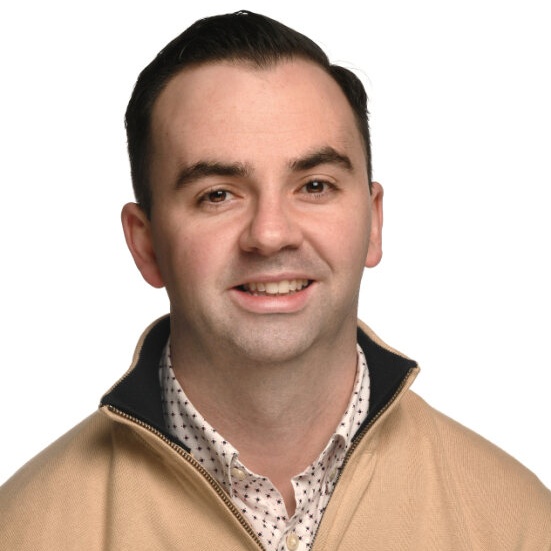
Ronan McCarthy is currently a Professor of Biomedical Sciences in the College of Health, Medicine and Life Sciences at Brunel University London. He leads a research programme on antibiotic resistance, antimicrobial discovery and biofilm regulation and disruption with a particular focus on Acinetobacter baumannii and Pseudomonas aeruginosa. This programme of work is supported by the Biotechnology and Biological Sciences Research Council, NC3Rs, Academy of Medical Sciences, Horizon 2020, British Society for Antimicrobial Chemotherapy, Innovate UK, Natural Environment Research Council and the Medical Research Council. He serves as an Editorial Board Member for npj Antimicrobials and Resistance.
The author declares that he does not have any relationships or affiliations that could be construed as a potential conflict of interest.